-
What is laser induced ignition?
Date posted:
-
-
Post Author
espadmin
1. Introduction
[GLOSS]Ignition[/GLOSS] is the process of starting [GLOSS]radical[/GLOSS] reactions until a self-sustaining flame has developed. Ignition was discussed in CF256, and characteristics of laser ignition are presented in CF269.
2. Laser-induced ignition
Generally, there are four basic mechanisms by which [GLOSS]laser[/GLOSS] radiation is able to ignite a combustible mixture:
· thermal initiation (thermal ignition)
· photochemical ignition
· resonant [GLOSS]breakdown[/GLOSS]
· non-resonant breakdown
2.1 Thermal initiation
In laser-induced thermal ignition there is no electrical breakdown of the gas. The laser radiation is used to heat up and to increase the temperature of the gas. Molecular bonds are cleaved and chemical reactions can take place. This ignition mechanism works well with solids because they generally absorb infrared radiation strongly.
2.2 Photochemical ignition
In resonant photochemical ignition laser photons, generally in the [GLOSS]UV[/GLOSS] range, are absorbed by the target molecules and cause them to dissociate into reactive radicals ([GLOSS]photolysis[/GLOSS]). If the radical production rate surpasses the recombination rate, chain branching reactions lead to ignition and finally to combustion. The energy necessary for a photochemical ignition is in the order of <1mJ. This process does not lead to direct heating or photo ionization and so it does not involve a breakdown of the gas. In fact, the technique can be used to ignite a combustible mixture if a sufficient amount of reactive radicals (in the order of 1017 atoms/cm3) within a sufficiently large volume is produced. The crucial factor for a successful ignition process is the radical concentration produced by absorption, and the volume in which the radicals are contained.
There are several disadvantages of photochemical ignition; since the photon energy in the visible and near-[GLOSS]IR[/GLOSS] range is smaller than the dissociation energy of most gases, this ignition mode is most effective using UV-laser sources which are very expensive.
2.3 Resonant breakdown
In resonant breakdown, a target molecule is dissociated by a non-resonant multi-photon dissociation process. The atoms produced are then ionized by a resonant multi-photon ionization process. Electrons that are generated in this second step (i.e. by resonant multi-photon absorption) absorb photons at any wavelength (continuum absorber). This third step leads to the formation of a [GLOSS]plasma[/GLOSS] (see [1] for details).
2.4 Non-resonant breakdown
This type is most similar to conventional spark ignition. Non-resonant breakdown is the most frequent ignition mode and is generally termed laser-induced spark ignition. In laser spark ignition a focused laser beam of irradiance in the order of 1010 W/cm2 (or laser photon flux in the order of 1029 photons/(cm2s)) is sufficient to generate a plasma spark either by multi-photon ionization or in an electron cascade process.
In the multi-photon ionization process a gas molecule or atom simultaneously absorbs a number of photons. If the photon energy absorbed is higher than the ionization potential of the molecule, the gas molecule is ionized. This process is important only at very short wavelengths (<1µm) or at very low pressure (<13 mbar), where collision effects are negligible. It becomes insignificant at visible and near [GLOSS]infrared[/GLOSS] wavelengths because the photon energy at these wavelengths is much smaller than the ionization potentials of most gases.
The electron cascade process requires the existence of initial electrons which are also called seed electrons. The electrons then absorb photons via the so-called inverse bremsstrahlung process, thereby increasing their kinetic energy. If the electrons gain sufficient energy, they ionize other gas molecules on impact, leading to an electron avalanche and breakdown of the gas. At high pressure (>132 mbar) and long wavelengths (>1 µm) this process usually dominates the generation of the plasma spark. The initial electrons necessary for the initiation of the electron cascade process can be generated by the multi-photon ionization process, if the laser irradiance is high enough. The presence of impurities, such as low-ionization-potential organic vapors or aerosol particles, can significantly facilitate the formation of the initial (seed) electrons.
The generated plasma spark of high temperature (in the order of 106 K) and pressure (in the order of 103 atm) leads to the formation of a rapidly expanding shock wave. It is the hot, laser-generated plasma that causes ignition.
Figure 1 shows a laser-induced spark produced in room air by non-resonant breakdown ([GLOSS]ND:YAG laser[/GLOSS], 10 mJ pulse energy, 1064 nm, 8 ns).
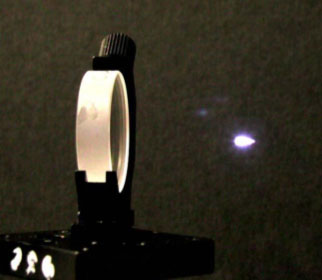
Figure 1: Image of a laser spark in room air (Picture taken by H. Kopecek). The laser (Q-switched Nd:YAG laser at 1064 nm was positioned to the left of this picture. The laser beam was focused through the window next to the spark.
Focusing a laser beam causes breakdown of the gas and produces a plasma with high temperature and pressure. The high pressure region induces a nearly spherical shock wave propagating away from the plasma kernel. A rarefaction wave characterized by a pressure smaller than the ambient pressure is generated. This leads to a complex flow pattern, and further vortical motions are developed. These vortical motions deform the flame kernel leading to a torus-like shape kernel and to the formation of the front lobe as illustrated in Figure 2.
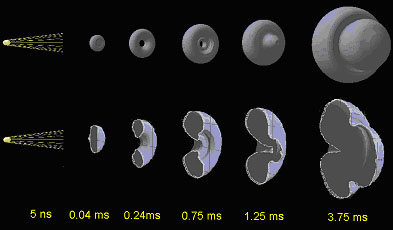
Figure 2: Flame kernel development of the torus and front lobe after laser energy deposition derived from LIF-measurements of [GLOSS]OH radical[/GLOSS]s. The laser beam was entering from the right (stoichiometric methane/air mixture, 1 bar, 296K, laser pulse energy 10 mJ at 1064 nm. Measurements conducted by D. Ruedisser, K. Iskra and Prof. T. Neger).
Figure 2 shows five images of the flame kernel development during the first 4 ms after the laser shot. The images were obtained from planar [GLOSS]laser induced fluorescence (LIF)[/GLOSS](PLIF) spectroscopic measurements.
In this case, PLIF was used to obtain temporally resolved two-dimensional cross-sections of the early stage flame kernel development by using the OH-radical as a coarse flame front marker. Excitation was performed at about 283 nm. The detected fluorescence signal was selected by a 10 nm bandwidth interference filter centered at 310 nm. The individual images were pasted together and, assuming rotational symmetry of the flame kernel, rendered to yield the images depicted in Figure 2.
The processes involved in laser induced ignition span several orders of magnitude in time, ranging from the nanosecond long energy deposition to the flame kernel development after several milliseconds.
3. Potential benefits of laser ignition
[GLOSS]Spark plug[/GLOSS]s which serve as ignition sources e.g. in [GLOSS]internal combustion engine[/GLOSS]s are associated with several problems. One of their main drawbacks is the periodic maintenance of the engine (especially for big, stationary devices). This fact leads to short service life times. In comparison to spark plugs, laser systems are contactless, therefore quenching effects due to heat losses can be avoided. Multi-point ignition can be performed and ignition locations can be chosen freely. Furthermore ignition timing and energy deposition can be controlled and programmed exactly. An important fact is the ability to ignite leaner mixtures with a laser source than with a spark plug. Leaner mixtures result in reduced temperatures, thereby minimizing [GLOSS]thermal NOx[/GLOSS] emissions. Yet no laser ignition system has made it to the market. Considerable research efforts are undertaken by academic institutions and engine manufacturers, however the costs of components and unsolved experimental problems pose severe problems to a widespread use of laser ignition (see Section 4 for details).
4. Future of laser ignition
Although laser ignition has been investigated widely [2-9] and numerous advantages have been discovered, first and foremost the lean mixture ignition capability and the possibility of free choice of the location, there is, up to now, no commercially available ignition system based on a laser.
This is predominantly due to the fact that lasers suitable for ignition of gases are more costly than electric spark plugs. Lasers are a potential alternative ignition source that might eventually replace electric spark plugs today in use [9]. Apart from the necessity to develop a suitably inexpensive, rugged and compact laser for series application, the optical window necessary to focus the laser beam into the engine remains the critical point. The window would have to withstand the high laser intensity. Also, it would have to remain transparent to the laser wavelength over a sufficiently long time span. This CF is about laser ignition of gases. Another active area of research is laser ignition of liquids and solid explosive materials [10].
Acknowledgements
The authors wish to acknowledge a fruitful cooperation with and valuable contributions from Dr. G. Herdin and J. Klausner, GE Jenbacher GmbH & CO OHG, Prof. E. Wintner and H. Kopecek, Vienna University of Technology, and Prof. T. Neger and K. Iskra, Graz University of Technology under FFF project grant 803050 and A3 project number 806238/7782.
Sources
[1] Forch B.; Miziolek A.W.; Laser-based ignition of H2/O2 and D2/O2 premixed gases near 243 nm: The first report of a deuterium isotope wavelength effect in laser ignition, Combustion Science and Technology 52:151 (1987).
[2] Phouc T.X.; Laser spark ignition: experimental determination of laser-induced breakdown thresholds of combustion gases, Optics Communications 175, 419-423 (2000).
[3] Weinberg F. J.; Wilson J. R.; A preliminary investigation of the use of focused laser beams for minimum ignition energy studies, Proc. Roy. Soc. Lond. A. 321, 41-52 (1971).
[4] Hickling R.; Smith W. R.; Combustion bomb tests of laser ignition; SAE Paper 740114, Society of Automotive Engineers, Detroit, Michigan, USA (1974).
[5] Dale J. D.; Smy P. R.; Clements R. M.; Laser ignited internal combustion engine – an experimental study; SAE Paper 780329, Society of Automotive Engineers, Detroit, Michigan, USA (1978).
[6] Ronney P. D.; Laser versus Conventional Ignition of Flames, Optical Engineering 33(2), 510-521 (1994).
[7]Lackner M.; Forsich C.; Winter F.; Kopecek H.; Wintner E.; In situ investigation of laser-induced ignition and the early stages of methane–air combustion at high pressures using a rapidly tuned diode laser at 2.55 µm; Spectrochimica Acta Part A: Molecular and Biomolecular Spectroscopy 59(23) 2997-3018 (2003).
[8] Kopecek, H.; Lackner, M.; Wintner, E.; Winter, F.; Laser-Stimulated Ignition in a Homogeneous Charge Compression Ignition Engine, SAE 2004 World Congress, paper No 2004-01-0937, Detroit, MI, USA, 2004.
[9]Kopecek H.; Wintner E.; Pischinger H.; Herdin G.; Klausner J.; Basics for a Future Laser Ignition System for Gas Engines; ICE-Vol.35-2, Paper No. 2000-ICE-316, 2000 ICE Fall Technical Conference ASME 2000, Preoria USA (2000).
[10] De Yong L.; Nguyen T.; Waschl J,; Laser Ignition of Explosives, Pyrotechnics and Propellants: A Review., DSTO Technical Report, DSTO-TR-0068, Australian Government Department of Defence, Canberra, Australia, (1995). http://www.dsto.defence.gov.au/corporate/reports/DSTO-TR-0068.pdf